ABOVE: © stock.adobe.com, Alpha Tauri 3D
In the 1980s, Steven Rosenberg, a cancer researcher and surgeon at the National Institutes of Health (NIH), started experimenting with harnessing and amplifying the immune system to fight cancer.1 He harvested lymphocytes that successfully infiltrated tumors and expanded them in a dish before infusing the T cells back into the patient.2 The results were encouraging, but there were two challenges: the tumor-infiltrating lymphocytes (TIL) failed to persist, and it was not always feasible to isolate sufficient T cells from patient tumors.3
Around the same time that Rosenberg assembled an army of the most successful T cells he could find, others developed strategies to arm these immune soldiers. The field of cell engineering took off in the 1980s, and scientists looked to introduce genes into cells to correct genetic disorders and tackle cancer. If scientists could engineer T cells then any old T cell would do and they wouldn’t require a large population of potentially limited tumor-specific T cells. Michel Sadelain, an immunologist at Memorial Sloan Kettering Cancer Center (MSKCC), focused his engineering efforts on T cells, despite a lack of interest from others in the field. He persevered and became a pioneer in T cell engineering, which opened the door to the future of cancer therapeutics: cell therapy, or living drugs.
Targeting solid tumors: a CAR T conundrum
T cells use an endogenous T cell receptor (TCR), a structure comprised of many different proteins, to identify foreign invaders and orchestrate cell death.4 The TCR is the business side of TIL therapy, which uses T cells enriched for specific TCR that, by nature of their discovery at the tumor site, successfully identify cancerous cells. However, the therapy’s success depends on the TIL-TCR repertoire, which may not target the optimal cancer marker. Furthermore, the reintroduced T cells eventually stall, requiring routine infusions to maintain T cell numbers.
The rise in genetic approaches over the last two decades allowed scientists to control the targeting and proliferation capabilities of T cells, regardless of the patient’s own TCR repertoire. To this end, Sadelain and others developed an artificial receptor called a chimeric antigen receptor (CAR). Today, there are several CAR models, but the first generation CAR consisted of an extracellular antigen binding domain tethered to a cluster of differentiation 3 zeta (CD3ζ) chain, a component of the endogenous TCR complex that couples antigen binding to a slew of intracellular signaling pathways that orchestrate an immune response, including cell death.5,6 Studies in mice demonstrated promising antitumor activity, but this early design only mimicked one facet of the endogenous T cell response. Following TCR activation, costimulatory molecules provide an added boost to sustain the attack. To give their CAR some extra fuel, Sadelain and his team added a CD28 domain, which enhanced T cell proliferation.7 This finding in 2002 ushered in the second-generation CAR, which soon advanced to clinical trials.
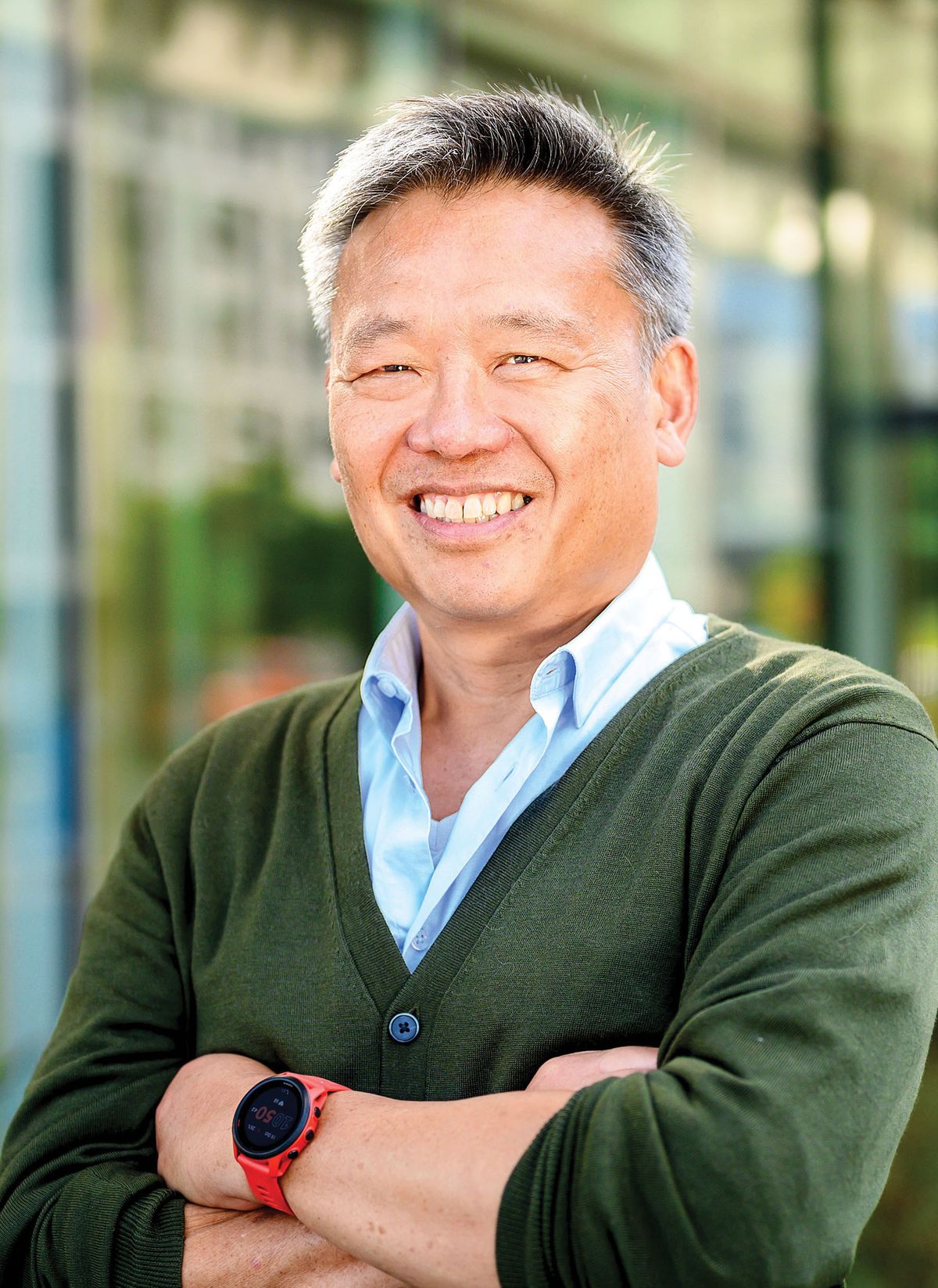
Cell-based immunotherapies reached an inflection point around 2010 when researchers at the NIH, MSKCC, and University of Pennsylvania released early clinical trial data on the efficacy of CAR T cells for treating certain blood cancers.5 “In a matter of a few months, each of these groups started reporting amazing, durable responses in patients with B cell cancers,” said Christopher Klebanoff, an oncologist and cellular therapist at MSKCC. Cancers that didn’t respond to chemotherapy and other targeted immunotherapies showed dramatic and long-lasting responses to the CAR T cell therapy.
These early results garnered the attention of academia and pharmaceutical companies, and more than a decade later, there are now six FDA approved CAR T cell therapies and more than a thousand ongoing clinical trials using some version of this technology. However, all six currently approved therapies target antigens that are expressed on B cells. “If you think of a pie chart that represents the distribution of different types of human cancers, maybe about five to six percent of all human cancers are malignant B cells of various types,” said Klebanoff. Although CAR T cell therapy got a fast start with its success in treating liquid cancers, it’s stalled for solid tumors.
CAR T cell therapy succeeded in liquid cancers for reasons related to its failures with solid tumors. Most approved CAR T cell therapies target the antigen CD19, which is expressed on all B cells.5 So, in addition to killing malignant cells, the therapy also wipes out healthy B cells. “The depletion of B cells really portends the challenge with trying to extend the technology to solid tumors,” said Klebanoff. “Rather remarkably, humans can do quite well without circulating B cells and not completely succumb to uncontrolled infections, which for me as a clinician, is actually as much a surprise as the consistency of the antitumor responses.”
B cells present a rather unique situation, but the risks of damaging healthy organs in pursuit of treating solid tumors are too high. A major bottleneck is identifying targets that are uniquely and homogeneously expressed by tumor cells, which are notoriously heterogeneous.3,5
Good targets aren’t the only things keeping cellular immunotherapies from reaching solid cancers. Tumors generate an inhospitable microenvironment that deters T cells, helping the cancer evade both endogenous and engineered immune attacks. Cancer cells also constantly mutate and adapt to their environments, so even if scientists discover tumor specific antigens and develop targeted therapies, cancer cells can simply stop expressing the antigen. These barriers to entry result in immunotherapies with limited specificity, reduced efficacy, and increased toxicity. For scientists developing novel cellular therapies, these are not mutually exclusive concerns; emerging strategies offer solutions for several of these challenges.
Upgrading CAR T operating systems
The solid tumor microenvironment suppresses the body’s immune system, camouflaging the cancerous cells from routine surveillance. To overcome the tumor’s perimeter defenses, researchers are searching for ways to load up T cells with tactical equipment.8
One strategy is to engineer CAR T cells to secrete stimulatory cytokines, which are small signaling molecules that play a role in immune modulation. A first choice was interleukin-12 (IL-12) due to its powerful proinflammatory activity and role in stimulating T cell function. Scientists observed strong antitumor activity in mice treated with IL-12; however, in humans, the cytokine caused severe toxicities, leading to hospitalization and in some cases, death.9,10
Sadelain and others wondered whether a smaller, more controlled, delivery of IL-12 could minimize toxicity, and engineered CAR T cells provided a convenient vehicle for testing their hypothesis. Indeed, armored CAR T cells that expressed IL-12 showed enhanced tumor cell death as well as resistance to T cell inhibition in mice.11,12 Several clinical trials are underway to test the efficacy of an IL-12 armored CAR and a variety of additional makes of armored CAR are in nonclinical and clinical stages of investigation.
We want to make the T cells smarter.
—Wendell Lim, University of California, San Francisco
Like other cancer therapies, CAR T cells can induce cytokine storms, and like native T cells, engineered T cells experience exhaustion—dysfunction resulting from their sustained attacks and the bombardment of immunosuppressive grenades from cancer cells.3,5 To put the brakes on toxicity and prevent burnout, scientists are developing remote controlled CAR systems that can be turned on or off in a variety of ways. A CAR designed to turn on or off in response to a drug, small molecule, or protease inhibitor may prevent cytokine storms from brewing.13-15
T cells engineered with an armored CAR or a remote-controlled CAR offer huge advancements for engineered cell therapies, giving scientists a means to boost an attack and limit toxicity. Despite this progress, scientists face additional challenges, including antigen escape and heterogeneity, which make solid cancers a moving target against which it is difficult to mount a sustained attack. The prevailing magic bullet mindset in cancer therapy limits researchers to a single antigen, which the cancer cell could stop expressing or which might not be specific to the tumor.16 Now, researchers look to upgrade T cells with multi-antigen recognition systems.
Wendell Lim, a systems biologist at the University of California, San Francisco, is pursuing a multi-antigen approach inspired by the sophisticated and multifaceted strategies that living cells use to recognize foreign antigens and mount a response. “We want to make the T cells smarter,” said Lim. Specifically, he wants to leverage the information processing capabilities of cells to develop therapeutic platforms that recognize combinatorial features of the tumor and then use this information to initiate a response.16
Cells engineered to detect a single antigen cannot differentiate between normal and cancerous cells. To improve specificity, Lim and others equip cells with multi-antigen recognition systems. Early versions of combinatorial CAR split the components of the synthetic receptor between two or three antigens so that CD3ζ was under the control of one antigen, and the costimulatory domains were under the control of other antigens, thus requiring at least two antigens to trigger the complete CAR T cell response.17,18
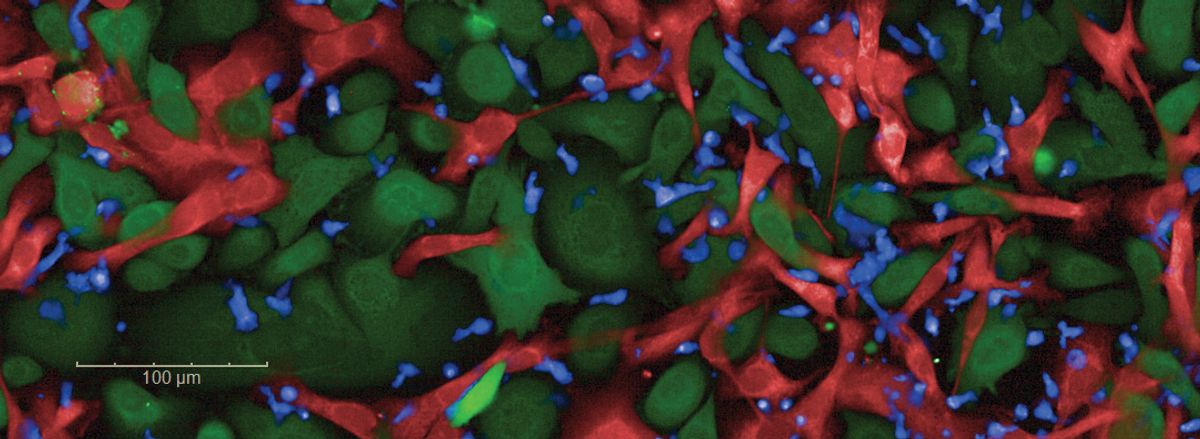
Lim’s team took another approach to programming multi-antigen recognition that’s based on algebra. “If there is not one single signal, can we use two or three signals so that by using Boolean logic, we can then differentiate cancer cells from normal tissue,” said Rogelio Hernández-López, a bioengineer at Stanford University, who previously worked as a postdoctoral researcher in Lim’s team.
To do this, Lim’s group designed synthetic Notch (synNotch) receptors that sense a specific antigen and in response, release a transcription factor that triggers the expression of genetically encoded cargo, such as a CAR, that targets a second antigen.19 This allowed the engineered T cells to use a different set of rules for recognition and killing. When tested in different mammalian cell lines, the logic-gated CAR specifically attacked dual antigen tumor cells while sparing single antigen cells.20
Lim and his team use the synNotch system to overcome several limitations of current cancer therapies. By sequentially linking multiple synNotch receptors, like a garland of daisies, upstream of CAR expression, the researchers added layers of recognition to the circuit to achieve a more precise and targeted attack.21 Similar to an armored CAR, the synNotch system can trigger cytokine release to not only guide the engineered CAR T cells towards the tumor site but also recruit bystander T cells.22 “The synNotch functions in the same cell, but as a different parallel pathway that helps stimulate and make the CAR T [cell] response stronger,” said Lim.
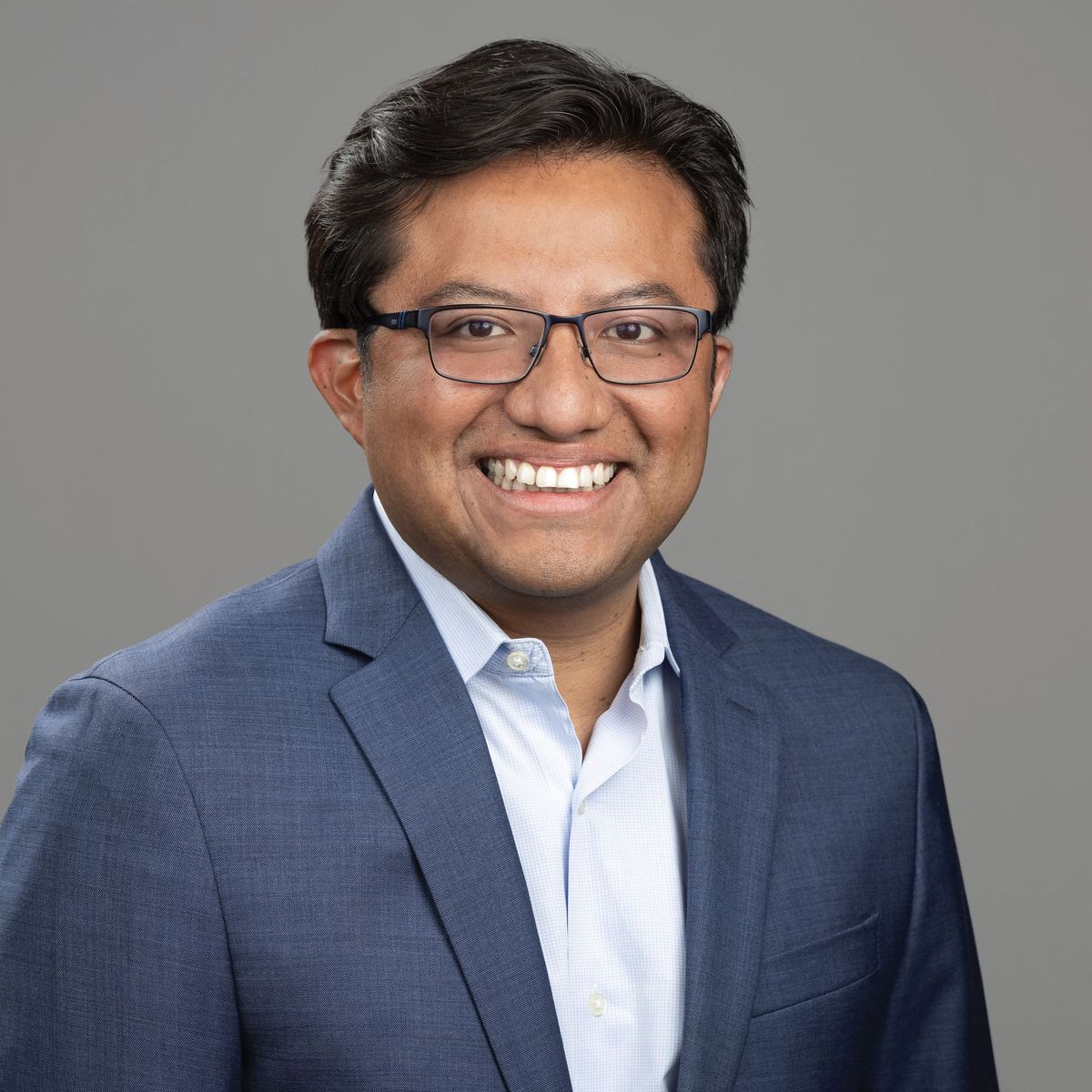
Although many antigens are expressed on both normal and cancerous cells, they are overexpressed on cancer cells. While he was working with Lim, Hernández-López engineered T cell circuits that sensed antigen density.23 In his system, the synNotch only triggered the expression of the CAR if the engineered T cell encountered a specific antigen threshold. This resulted in an ultrasensitive system that discriminated cancer cells from normal cells. In his lab at Stanford University, Hernández-López is using patient-derived organoids and immunocompetent mouse models to test the system with more complex tumors.
CAR and logic-gated systems are modular and, when multiplexed, scientists can build multi-antigen recognition systems that serve as GPS systems to guide T cells for therapeutic applications. The possible combinations driving recognition and killing are seemingly endless. Hernández-López is looking beyond the confines of the engineered cell for other systems that can join the attack. “Encoding these different functions into a single cell is going to be very challenging, so I’m more excited about how we use these engineered cells to kickstart a function or engage with other cells in the immune system so that together they can collectively have better efficacy,” said Hernández-López.
Understanding CAR T vs TCR ApproachesLiving drugs get a boost from emerging engineering strategies that focus on safety and specificity. |
![]() © lisa clark (1) A chimeric antigen receptor (CAR) is a modular, synthetic receptor that endows T cells with different targeting abilities. Several emerging engineering approaches aim to improve the safety, specificity, and efficacy of CAR therapies. (2) Engineered CAR T cells expressing therapeutic payloads can transform the immunosuppressive environment of solid tumors, such as through producing and secreting proinflammatory cytokines. (3) CAR T cells that perform combinatorial logic operations help cells discriminate between healthy and cancerous cells and hone their attack, thus reducing toxicity. For example, activation of a synthetic Notch (synNotch) receptor that binds one antigen can trigger the expression of a CAR targeting another antigen. (4) A CAR controlled remotely via drugs and other small molecules puts the brakes on T cell activation and prevents exhaustion. |
![]() © lisa clark (5) The T cell receptor (TCR) is a protein complex that recognizes different peptides and triggers T cell activation. A TCR genetically engineered to bind specific peptide-HLA complexes can improve targeting specificity. (6) Soluble TCR and TCR mimics are small proteins that bind peptides and engage with the CD3ε subunit of the endogenous TCR to create a synthetic immunological synapse. (7) A T cell antigen coupler (TAC) and a TCR fusion construct (TRuC) also engage endogenous TCR signaling, but, similar to a CAR, they bind to tumor antigens. This can increase receptor sensitivity while reducing cytotoxicity and T cell exhaustion observed with a CAR. (8) Synthetic TCR antigen receptor/HLA-independent TCR (STAR/HIT) constructs retain the full complex of CD3 signaling but also have high antigen sensitivity via the fusion of an antibody binding domain. This hybrid receptor design combines the strengths of a CAR and a TCR. |
TCR therapies: targeting the peptide buffet
In the early 2000s, as part of her medical specialty training, Johanna Olweus, a physician and immunologist at Oslo University Hospital, completed a brief stint in pathology. She learned about leukemia diagnostics and how clinicians used two main markers to determine whether a sample was a myeloid or a lymphoid leukemia. Both markers were intracellular. “I then thought that maybe those could be very good targets for cancer immunotherapy,” said Olweus.
The binding domain of a CAR is based on antibodies and therefore targets cell surface antigens. However, nearly 90% of proteins in the human proteome, including the markers Olweus had her sights set on, live exclusively in intracellular compartments that are inaccessible to a CAR.24 In contrast, the endogenous TCR can target both intracellular and membrane-bound proteins, thanks to complexities in the binding domain of the receptor.
It’s not one TCR fits all; that’s for sure.
—Johanna Olweus, Oslo University Hospital
Atop the TCR’s CD3ζ chains and nestled between CD3γ, CD3δ, and CD3ε subunits sit TCRα and TCRβ protein chains that interact with each other to recognize snippets of a protein.4 Inside cells, proteins continuously degrade and recycle via the proteasome, which Klebanoff described as the great trash compactor of cells. Proteins get chopped up into smaller peptide sequences, which get scooped up by a major histocompatibility complex (MHC) (referred to as the human version, human leukocyte antigens (HLA)), which is a complex of proteins bound for the membrane. Once the HLA samples the peptide buffet, it shuttles these fragments to the surface of cells for detection by patrolling T cells, which use these peptide hors-d’œuvres to determine whether they originate from normal or abnormal proteins.
“The reason that I’m so excited by TCR therapies is that it increases the number and types of proteins that might be targeted by immune cells, probably by an order of magnitude or more than current CAR-based therapies,” said Klebanoff.
Variability in the sequences of the outermost domains of TCRα/β chains and their combinatorial possibilities allow the TCR to bind different peptide-HLA complexes.4 By the late 1990s, scientists found ways to select human T cells expressing TCR with a desired binding profile and transfer the TCR genes to new T cells to genetically redirect their attacks.
While combing through the TCR profiles of patient TIL, Rosenberg and his colleagues identified a TCR specific for a peptide associated with melanoma antigen recognized by T cells 1 (MART-1).25 They cloned the gene coding for the TCR and transferred it to lymphocytes extracted from patients with metastatic melanoma before reintroducing the genetically engineered cells.26 This was the first demonstration that human T cells engineered to express an exogenous TCR conferred cancer regression.
Despite all of the potential peptides in the cellular sea for a TCR to latch onto, there’s a catch. For a TCR to work, it needs not only a specific target, but also that the target is attached to a compatible HLA. In humans, HLA types are similar to blood groups, but instead of four possibilities, there are thousands of HLA molecules, and each person has a unique set of HLA types. “The HLA locus is actually the most diverse genetic locus we have,” said Olweus. Different genes in the HLA locus determine which peptides the complex will load and present. This level of diversity is great in a pandemic when at least some of the population would effectively capture pathogenic peptides and mount an adequate immune response. “If you’re designing a TCR for cancer immunotherapy, you will have to design one TCR for one person and another for another person,” said Olweus.
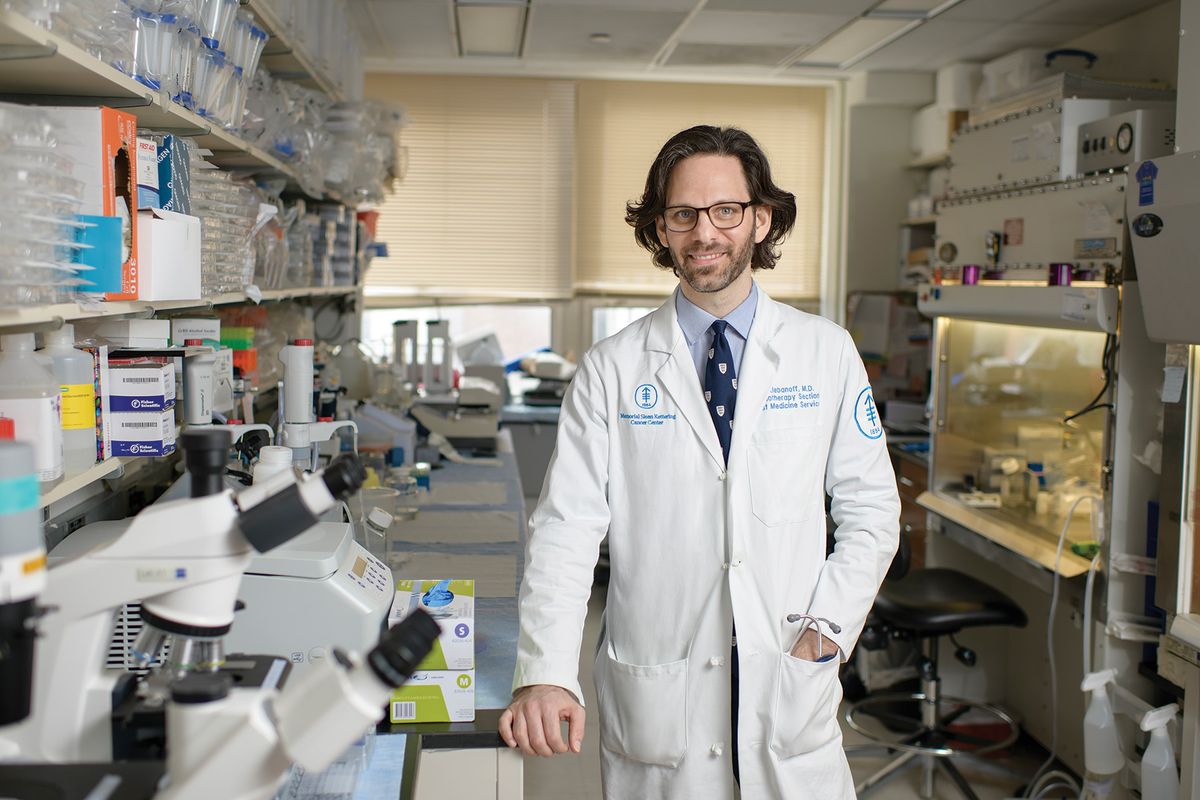
To determine whether a patient might benefit from a particular engineered TCR therapy, clinicians need to run two tests to determine whether the patient’s cancer cells express the target antigen and the specific tissue type. Luckily, some HLA types are more common; for example, one study found that approximately 35 percent of African American individuals and 50% of White individuals had the HLA-A2 subtype.27 “It’s not one TCR fits all; that’s for sure,” said Olweus. However, with a few carefully selected HLA types, you can cover most individuals. “The goal is to create a library of vetted TCR that have the perfect mixture of potency and specificity and that are compatible with different HLA types so that we can fill out the pie chart of trying to accommodate as many patients as possible,” said Klebanoff.
In the mid-2000s, Olweus revisited her idea of developing a cancer immunotherapy targeting an intracellular marker. She noticed the strong immune response elicited by organ rejection, a result of an HLA mismatch. Olweus also read that if cancer develops in the transplanted organ and patients cease taking immunosuppressive drugs, the subsequent immune response that triggers organ rejection can also eliminate the cancer, even when it has metastasized beyond the transplanted organ. “This showed me that the T cells definitely can cure cancer, even solid cancer, if they have good enough targets,” said Olweus.
Returning to the leukemia diagnostics panel, she knew that terminal deoxynucleotidyl transferase (TdT) was expressed in 80-90 percent of B cell and T cell type lymphoid leukemias.28 Like other markers, an HLA complex presents TdT peptide fragments to the immune system. However, since these are recognized by passing T cells as self-antigens, no alarms go off because the thymus eliminates T cells harboring a TCR that recognize self-antigens.
Although this security screen protects people from autoimmunity, it also prevents scientists from exploiting normal proteins that could otherwise be great targets for cancer immunotherapies. To circumvent this, Olweus wondered whether she could engineer T cells to recognize the TdT peptides as foreign and trigger a powerful and targeted immune response similar to what is seen with organ rejection. After demonstrating the feasibility of targeting self-antigens in the context of a foreign HLA, her team set out to target TdT.29
This showed me that the T cells definitely can cure cancer, even solid cancer, if they have good enough targets.
—Johanna Olweus, Oslo University Hospital
For their next experiment, they identified patients whose cancer cells presented TdT on HLA-A2 platters.30 Then the team collected T cells from healthy donors that lacked HLA-A2 and screened them for a TCR that identified the TdT-HLA-A2 complex found in the patients’ cancer cells. In this scenario, because a foreign HLA served up the TdT self-peptide, the T cells recognized the peptide as foreign and initiated an attack. Next, they sequenced positive hits, introduced the donor-derived TCR into third party T cells, and tested them against human cancer cells in a dish and in mouse models with patient-derived leukemia. Their engineered TCR T cells killed cancerous cells while sparing normal lymphocytes.
“I’m very excited that we’re getting closer to testing our T cell receptors in the clinic,” said Olweus. Upcoming clinical trials will examine the therapeutic potential of this engineered TCR in patients with leukemia. Still, translating engineered TCR T cell therapies into therapy is costly and requires HLA matching. To overcome these limitations, scientists are developing off-the-shelf therapeutics that interact with the TCR, combining the benefits of TCR and CAR approaches.
Off-the-shelf CAR-TCR therapies
In January 2022, the FDA approved the first TCR-based therapeutic.31 Approved for the treatment for uveal melanoma, the therapy targets the cancer antigen gp100 presented on HLA-A2. Although it sounds similar to Olweus’ TCR T cells, this therapy comes from a new class of biologics designed to engage a patient’s native T cells.
The treatment is based on a platform called immune-mobilizing monoclonal T cell receptor against cancer (ImmTAC).32 Scientists used protein engineering to develop a soluble, recombinant protein version of the variable TCRαβ binding region and fused it to a monoclonal antibody that binds to the CD3ε domain of an endogenous TCR. Ultimately, the soluble TCR creates a synthetic immunological synapse to bridge cancer cells that express the antigen with T cells that do not normally have specificity for the target, thus recruiting more immune foot soldiers to the war.4 A soluble TCR provides an alternative approach to redirecting a patient’s native T cells towards a common cancer target that doesn’t require engineering cells outside of the body and reintroducing them. However, this isn’t the only emerging strategy that engages and co-opts the natural TCR signaling machinery.
2024 is going to be the year of the TCR.
—Christopher Klebanoff, Memorial Sloan Kettering Cancer Center
TCR mimics use a similar approach to bind to the endogenous TCR, but they have an antibody design to bind to the peptide-HLA.4,33 Therefore, TCR mimics can benefit from the well trodden path of monoclonal antibody production, such as phage display, to generate a huge diversity of candidate binding domains on an antibody. In theory, this should streamline the development of proteins that bind the peptide-HLA complex. However, Klebanoff noted, “HLA is this incredibly elegant evolutionary process that has been with us for 350 million years.” TCR and HLA have had a long time to develop an intricate relationship. “The width and the structure of the TCR has evolved in a way that allows it to have a maximum breadth, sort of width, to sample enough of the peptide and enough of the HLA to drive specificity,” he added. In contrast, most antibodies have a narrower bite, which limits their specificity for a target peptide-HLA. This can result in the TCR mimic docking at unintended targets, increasing the risk of cross reactivity. This has led scientists to mine for rare, ultrasensitive antibodies that may improve the specificity of these TCR engagers.4
The TCR-HLA relationship is a double-edged sword. While it opens the door to more cancer targets, especially for solid tumors, it requires patient HLA matching, thus excluding patients who present the target cancer peptide on a different HLA platter. To develop HLA-independent approaches that still benefit from TCR prowess in immune warfare, scientists have started to develop hybrid designs. Like a CAR, they use an antigen binding domain, but unlike a CAR, they plug into a TCR to tap into its signaling machinery. A few hybrid designs have emerged, and they differ in how the antigen binding domain is wired up to the TCR.4
T cell antigen couplers (TAC) and TCR fusion constructs (TRuC) hook up their antigen binding domains to the CD3ε domain of an endogenous TCR.34,35 Because these receptors do not have the intrinsic capacity to signal on their own and depend on the TCR, they may prevent accelerated T cell exhaustion and elevated cytokine release observed with CAR designs.4 Klebanoff noted that this is due in part to differences in the signaling cadence of the two receptors.
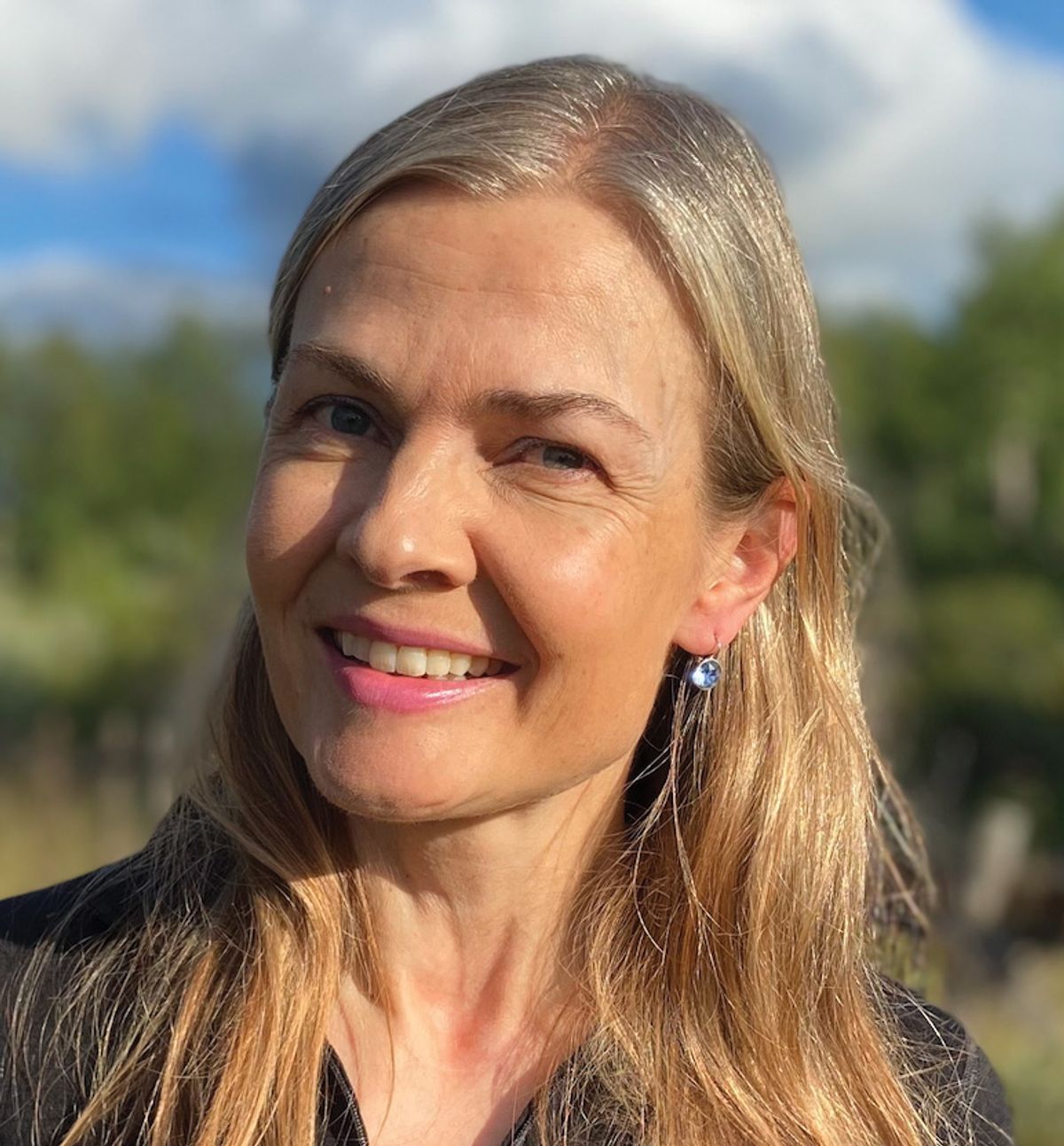
“A CAR is sort of like a Tesla. It goes [from] zero to 60 in like two seconds,” said Klebanoff. In contrast, TCR activity is more gradual. In the context of highly potent cell therapies, CAR’s rapid activation can lead to a sudden release of cytokines, which causes toxicities. The hope is that a more gradual release of cytokines translates to lower toxicities.
Many tumor antigens are expressed in low numbers and cancer cells even downregulate antigens to evade antigen-targeted treatments. Another limitation of a CAR relative to a TCR is the antigen binding threshold required to trigger cytolysis. A CAR typically requires thousands of molecules whereas an endogenous TCR recognizes much smaller levels of peptide-HLA. Some estimates go as low as one to three peptides per cell.36 This raises the possibility that a hybrid receptor could improve the targeting of a CAR towards low antigen cancer cells.
Synthetic TCR antigen receptor (STAR) and HLA-independent TCR (HIT) are synthetic receptors generated using genome editing techniques to swap out the variable TCRαβ binding region of a TCR with elements of a CAR binding domain.37,38 Sadelain and his team found that their HIT receptor outperformed a CD28-based CAR, the most sensitive version, when it came to targeting low abundance antigens, a feature they attributed to the TCR complex. “It actually could be the best of both worlds: a more sensitive antigen receptor and an antigen receptor that has a lower potential for toxicities related to cytokine release,” said Klebanoff.
Living drugs of the future
It has been nearly 40 years since Rosenberg set out to harness a patient’s immune cells to fight cancer. In the years that followed, advances in gene editing and cell engineering gave scientists the ability to engineer receptors and antibodies that boost the endogenous immune system. Now, these living drugs are a clinical reality for many patients. Over the last decade, thousands of patients received CAR T cell therapies, and thousands of clinical trials are under way.
Data coming from nonclinical and clinical studies using TCR T cells and TCR-based therapeutics are promising, but still limited. “TCR T cell therapy is still in its infancy,” said Olweus. In contrast to CAR T cell therapies, which have found their ways into more than 1,000 ongoing clinical trials, there are only 20-30 published TCR T cell clinical trials, all of which are from phase I or II studies.39 Klebanoff said that the future of TCR-based therapies is bright. He added, “2024 is going to be the year of the TCR.” He anticipates that the FDA will approve both TIL for treatment of advanced melanoma* and an engineered TCR T cell therapy targeting the antigen MAGE-A4 for the treatment of synovial sarcoma.40
*Note: On February 16, 2024, post Klebanoff's interview, the FDA approved Amtagvi (lifileucel) for the treatment of metastatic melanoma. This is the first TIL therapy approved by the agency and the first immune cell therapy for treating solid tumors.
- Rosenberg SA, et al. A new approach to the adoptive immunotherapy of cancer with tumor-infiltrating lymphocytes. Science. 1986;233(4770):1318-1321.
- Rosenberg SA, et al. Use of tumor-infiltrating lymphocytes and interleukin-2 in the immunotherapy of patients with metastatic melanoma. N Engl J Med. 1988;319:1676-1680.
- Irvine DJ, et al. The future of engineered immune cell therapies. Science. 2022;378(6622):853-858.
- Klebanoff CA, et al. T cell receptor therapeutics: Immunological targeting of the intracellular cancer proteome. Nat Rev Drug Discov. 2023;22:996-1017.
- Levin AG, et al. CAR T cells: Building on the CD19 paradigm. Eur J Immunol. 2021;51(9):2151-2163.
- Eshhar Z, et al. Specific activation and targeting of cytotoxic lymphocytes through chimeric single chains consisting of antibody-binding domains and the gamma or zeta subunits of the immunoglobulin and T-cell receptors. Proc Natl Acad Sci USA. 1993;90(2):720-724.
- Maher J, et al. Human T-lymphocyte cytotoxicity and proliferation directed by a single chimeric TCRζ/CD28 receptor. Nat Biotechnol. 2002;20(1):70-75.
- Rafiq S, et al. Engineering strategies to overcome the current roadblocks in CAR T cell therapy. Nat Rev Clin Oncol. 2019;17(3):147-167.
- Trinchieri G. Interleukin-12: A cytokine produced by antigen-presenting cells with immunoregulatory functions in the generation of T-helper cells type 1 and cytotoxic lymphocytes. Blood. 1994;84(12):4008-4027.
- Leonard JP, et al. Effects of a single-dose interleukin-12 exposure on interleukin-12-associated toxicity and interferon-???? production. Blood. 1997;90(7):2541-2548.
- Yeku OO, et al. Armored CAR T cells enhance antitumor efficacy and overcome the tumor microenvironment. Sci Rep. 2017;7(1):10541.
- Pegram HJ, et al. Tumor-targeted T cells modified to secrete IL-12 eradicate systemic tumors without need for prior conditioning. Blood. 2012;119(18):4133-4141.
- Jan M, et al. Reversible ON- and OFF-switch chimeric antigen receptors controlled by lenalidomide. Sci Transl Med. 2021;13(575):eabb6295.
- Wu C-Y, et al. Remote control of therapeutic T cells through a small molecule-gated chimeric receptor. Science. 2015;350(6258):eaab4077.
- Juillerat A, et al. Modulation of chimeric antigen receptor surface expression by a small molecule switch. BMC Biotechnol. 2019;19:44.
- Allen GM, Lim WA. Rethinking cancer targeting strategies in the era of smart cell therapeutics. Nat Rev Cancer. 2022;22(12):693-702.
- Wilkie S, et al. Dual targeting of ErbB2 and MUC1 in breast cancer using chimeric antigen receptors engineered to provide complementary signaling. J Clin Immunol. 2012;32(5):1059-1070.
- Kloss CC, et al. Combinatorial antigen recognition with balanced signaling promotes selective tumor eradication by engineered T cells. Nat Biotechnol. 2013;31(1):71-75.
- Morsut L, et al. Engineering customized cell sensing and response behaviors using synthetic notch receptors. Cell. 2016;164(4):780-791.
- Roybal KT, et al. Precision tumor recognition by T cells with combinatorial antigen-sensing circuits. Cell. 2016;164(4):770-779.
- Williams JZ, et al. Precise T cell recognition programs designed by transcriptionally linking multiple receptors. Science. 2020;370(6520):1099-1104.
- Allen GM, et al. Synthetic cytokine circuits that drive T cells into immune-excluded tumors. Science. 2022;378(6625):eaba1624.
- Hernández-López RA, et al. T cell circuits that sense antigen density with an ultrasensitive threshold. Science. 2021;371(6534):1166-1171.
- Thul PJ, et al. A subcellular map of the human proteome. Science. 2017;356(6340):eaal3321.
- Kawakami Y, et al. Cloning of the gene coding for a shared human melanoma antigen recognized by autologous T cells infiltrating into tumor. Proc Natl Acad Sci USA. 1994;91(9):3515-3519.
- Clay TM, et al. Efficient transfer of a tumor antigen-reactive TCR to human peripheral blood lymphocytes confers anti-tumor reactivity. J Immunol. 1999;163(1):507-513.
- Ellis JM, et al. Frequencies of HLA-A2 alleles in five US population groups: Predominance of A*02011 and identification of HLA-A*0231. Human Immunol. 2000;61(3):334-340.
- Drexler HG, et al. Incidence of TdT positivity in cases of leukemia and lymphoma. Acta Haematol. 1986;75(1):12-17.
- Kumari S, et al. Alloreactive cytotoxic T cells provide means to decipher the immunopeptidome and reveal a plethora of tumor-associated self-epitopes. Proc Natl Acad Sci USA. 2013;111(1):403-408.
- Ali M, et al. T cells targeted to TdT kill leukemic lymphoblasts while sparing normal lymphocytes. Nat Biotechnol. 2021;40:488-498.
- Nathan P, et al. Overall survival benefit with tebentafusp in metastatic uveal melanoma. N Engl J Med. 2021;385:1196-1206.
- Liddy N, et al. Monoclonal TCR-redirected tumor cell killing. Nat Med. 2012;18:980-987.
- Dao T, et al. Therapeutic bispecific T cell engager antibody targeting the intracellular oncoprotein WT1. Nat Biotechnol. 2015;33:1079-1086.
- Helsen CW, et al. The chimeric TAC receptor co-opts the T cell receptor yielding robust anti-tumor activity without toxicity. Nat Commun. 2018;9:3049.
- Baeurele PA, et al. Synthetic TRuC receptors engaging the complete T cell receptor for potent anti-tumor response. Nat Commun. 2019;10:2087.
- Purbhoo MA, et al. T cell killing does not require the formation of a stable mature immunological synapse. Nat Immunol. 2004;5:524-530.
- Liu Y, et al. Chimeric STAR receptors using TCR machinery mediate robust responses against solid tumors. Sci Transl Med. 2021;13(586):eabb5191.
- Mansilla-Soto J, et al. HLA-independent T cell receptos for targeting tumors with low antigen density. Nat Med. 2022;28:345-352.
- Baulu E, et al. TCR-engineered T cell therapy in solid tumors: State of the art and perspectives. Sci Adv. 2023;9(7):eadf3700.
- Hong DS, et al. Autologous T cell therapy for MAGE-A4+ solid cancers in HLA-A*02+ patients: A phase I trial. Nat Med. 2023;29:104-114.
Membership Open House!
Enjoy OPEN access to Premium Content for a limited timeInterested in exclusive access to more premium content?