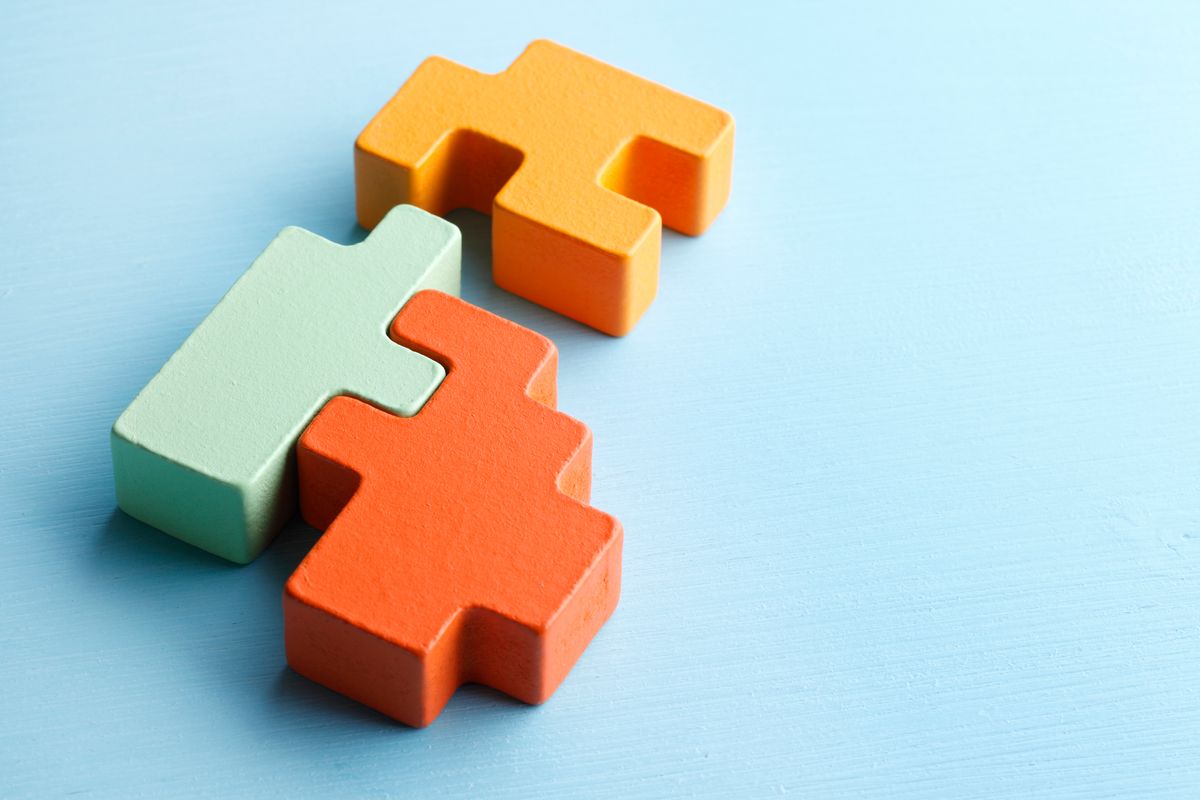
Stay up to date on the latest science with Brush Up Summaries.
What Is Click Chemistry?
Click chemistry is a class of fast and simple reactions that scientists use to connect two molecules together. It includes many synthesis strategies that fall under the umbrella of click reactions, which researchers design by taking inspiration from high yield reactions that occur naturally.1 Although the principles of click chemistry extend beyond biological applications, its overlap with bioorthogonal reactions that occur quickly and selectively in biological environments is particularly useful for scientists who create and detect biomolecules.2
The 2022 Nobel Prize in Chemistry was awarded to Carolyn Bertozzi, Morten Meldal, and Barry Sharpless for the development of click chemistry and bioorthogonal chemistry. Each laureate played a crucial role in the click chemistry field, with Bertozzi advancing this approach into living systems by developing bioorthogonal reactions, click chemistry that functions without disrupting the cell.3
How Does Click Chemistry Work?
Click chemistry includes many types of reactions, such as copper-catalyzed azide-alkyne cycloaddition and various strain-promoted reactions.4 The vast class of reactions encompassed by click chemistry share several key characteristics.1
- Selective and modular: Click reactions connect separate subunits together to create large amounts of a desired product while producing minimal to no harmful byproducts.
- One-pot synthesis: Click reactions typically occur in a single reactor without lengthy separation and purification processes, saving time and resources.
- Simple conditions, materials, and reagents: Ideally, click reactions are insensitive to water and oxygen, and use benign or easily removed solvents, if any.
- Thermodynamically favorable: Click reactions are often described as spring-loaded, meaning that they occur quickly and irreversibly, with high yield and reaction specificity.
These criteria are part of the reason click chemistry is adaptable across many synthetic applications. It allows researchers to create intricate molecular structures from a wide range of substrates.4
When scientists use synthetic chemistry for biological applications, the rules that govern click chemistry have higher stakes. In living systems, reaction products need to be physiologically stable, and there is little leeway for byproduct toxicity.4
Bioorthogonal chemistry encompasses a class of click reactions that are both fast and biologically inert, meaning that they do not interfere with biological processes. Bioorthogonal reactions allow scientists to examine biomolecules such as nucleic acids, lipids, and glycans in living systems, helping researchers label, isolate, and target molecules in complex biological environments.2
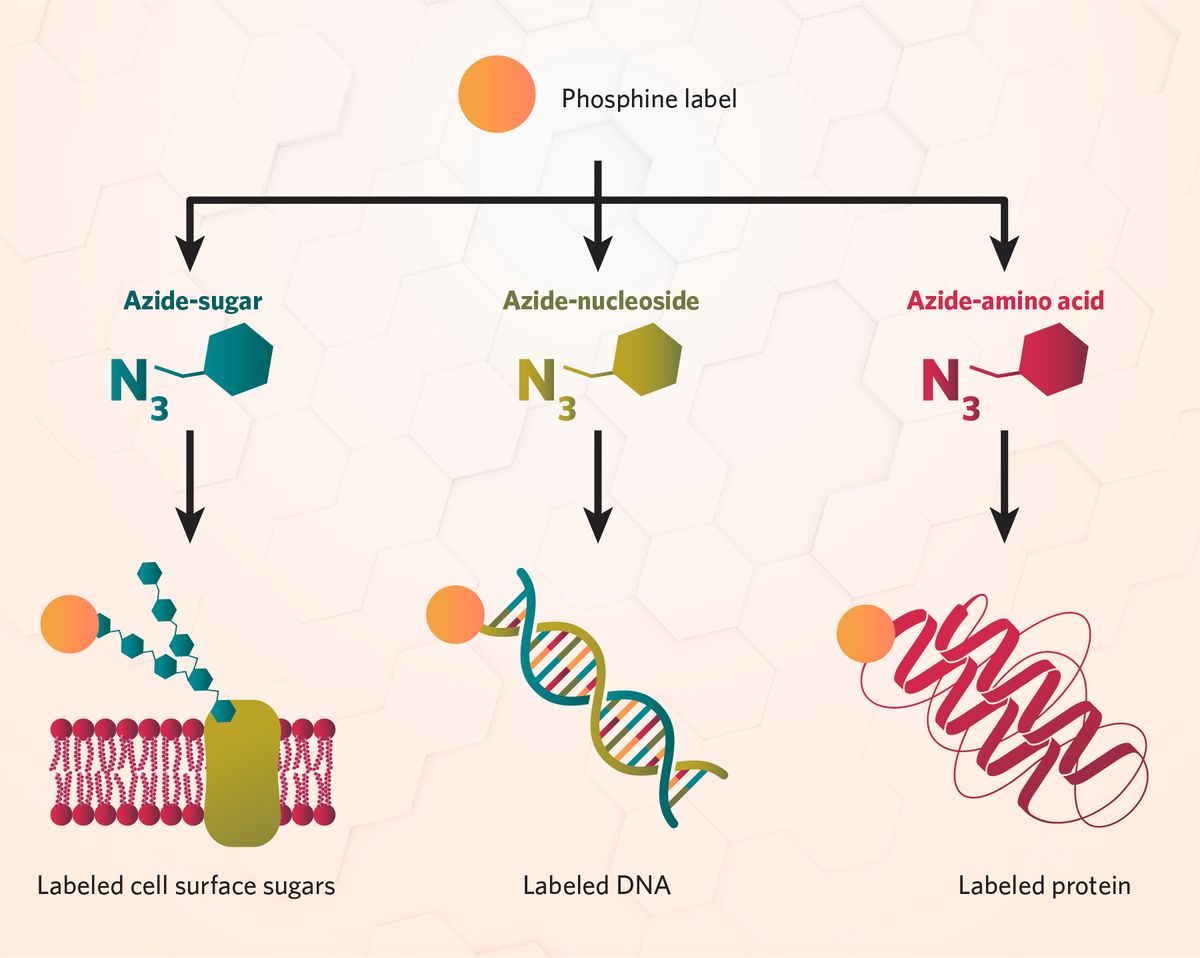
How Scientists Use Click Chemistry and Bioorthogonal Chemistry
Cellular imaging and multiplexing
Click reactions enable the selective labeling of biomolecules for cellular imaging. Traditional labeling methods often involve perturbing cellular processes or using large, cumbersome bioconjugates such as fluorescent protein tags. In contrast, bioorthogonal chemistry allows scientists to introduce small, non-disruptive chemical tags into biological systems, such as a nucleoside for DNA labeling, and detect the tag with a bioorthogonal imaging label, such as a fluorescent dye.2
These tools facilitate live cell imaging and purification techniques without altering the natural behavior of the target biomolecules, including proteins, nucleic acids, glycans, and lipids. With multiplexing, bioorthogonal chemistry also allows simultaneous detection of multiple biomolecules by using distinct chemical tags in the same experiment.5
Diagnostics
Scientists use click chemistry to improve diagnostic imaging. For example, because different carbohydrates interact with specific cell surface receptors, they are ideal candidates for creating glycoprobes through click reactions. Fluorescent glycoprobes that include a carbohydrate for target recognition and a fluorescent tag for detection are well established, and researchers have developed variations such as click-conjugated glyconanoparticles and electrochemically active glycoprobes.6,7 Scientists also use click chemistry to improve nuclear imaging probes, such as those used in positron emission tomography, or PET scan imaging.8,9
Drug delivery, PROTAC, and LYTAC
Scientists leverage click chemistry in precision medicine to connect therapeutic agents with targeting molecules. This allows researchers to design carriers such as antibody-drug conjugates that selectively deliver drugs to specific tissues or cells, improving therapeutic efficacy and minimizing off-target effects.4
Another prominent example of click chemistry for drug delivery is the rapidly expanding therapeutic field of targeted protein degradation, which includes proteolysis-targeting chimeras (PROTACs) and its younger cousin lysosome-targeting chimeras (LYTACs).4,10,11
PROTAC therapeutics recruit ubiquitin ligases to unwanted proteins, targeting them for proteasomal degradation, while LYTACs deliver the target protein to the lysosome for degradation. Because these bifunctional molecules are more specific than traditional enzymatic inhibitors and they highjack endogenous degradation pathways, they often function at lower doses and reach previously undruggable proteins with inaccessible catalytic sites.10,11
Targeted degradation is a common thread connecting current bioorthogonal chemistry approaches to up and coming drug delivery strategies. Click reactions facilitate PROTAC synthesis and bioorthogonality inspires targeted degradation therapeutics as a whole, including the targeted sialidases and mucinases created by Bertozzi’s research team for anticancer applications.12-14
- Kolb HC, et al. Click chemistry: Diverse chemical function from a few good reactions. Angew Chem Int Ed Engl. 2001;40(11):2004-21.
- Sletten EM, Bertozzi CR. Bioorthogonal chemistry: Fishing for selectivity in a sea of functionality. Angew Chem Int Ed. 2009;48(38):6974-6998.
- The Nobel Prize in Chemistry 2022. NobelPrize.org.
- Scinto SL, et al. Bioorthogonal chemistry. Nat Rev Methods Primers. 2021;1(1).
- Sletten EM, Bertozzi CR. A bioorthogonal quadricyclane ligation. J Am Chem Soc. 2011;133(44):17570-17573.
- He X, et al. Carbohydrate CuAAC click chemistry for therapy and diagnosis. Carbohydr Res. 2016;429:1-22.
- Zhang J, et al. Remote light-controlled intracellular target recognition by photochromic fluorescent glycoprobes. Nat Commun. 2017;8(1).
- Nwe K, Brechbiel MW. Growing applications of “Click Chemistry” for bioconjugation in contemporary biomedical research. Cancer Biother Radiopharm. 2009;24(3):289-302.
- Sarrett SM, et al. Inverse electron demand Diels–Alder click chemistry for pretargeted PET imaging and radioimmunotherapy. Nature Protoc. 2021;16(7):3348-3381.
- Sakamoto KM, et al. Protacs: Chimeric molecules that target proteins to the Skp1–Cullin–F box complex for ubiquitination and degradation. PNAS. 2001;98(15):8554-8559.
- Banik SM, et al. Lysosome-targeting chimaeras for degradation of extracellular proteins. Nature. 2020;584(7820):291-297.
- Zhang Q, et al. Designing bioorthogonal reactions for biomedical applications. Research (Wash D C). 2023;6:0251.
- Gray MA, et al. Targeted glycan degradation potentiates the anticancer immune response in vivo. Nat Chem Biol. 2020;16(12):1376-84.
- Pedram K, et al. Design of a mucin-selective protease for targeted degradation of cancer-associated mucins. Nat Biotechnol. [published online: August 03, 2023].