Article reviewed by George Heath, PhD from the University of Leeds.
Stay up to date on the latest science with Brush Up Summaries.
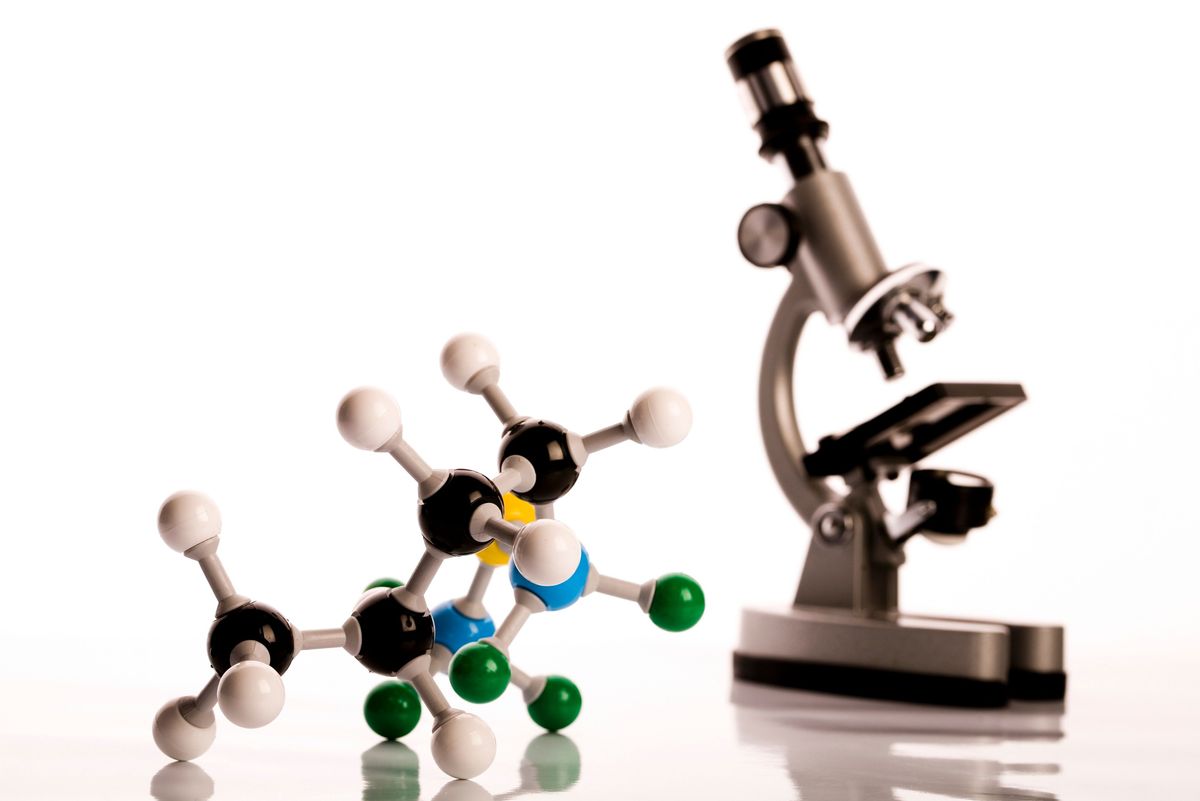
What Is Atomic Force Microscopy?
Atomic force microscopy (AFM) is a powerful technique that enables surface ultrastructure visualization at molecular resolution.1 Besides three-dimensional (3D) surface characterization, this analytical tool also provides information about a sample’s physical properties, including its surface hydrophobicity, molecular interactions, surface charges, and mechanical properties.
In 1986, scientists at IBM and Stanford University collaborated to develop AFM.2 The three scientists behind this revolutionary invention were Calvin Quate, Gerd Binnig, and Christoph Gerber.
AFM Working Principle and Instrumentation
AFM gathers useful information by feeling a surface with a probe to sense small variations. This is analogous to touching a surface with fingers but on a much smaller scale. Scientists use AFM to visualize surface properties such as roughness in different sample types including glass, ceramic, polymer, and biological substances (e.g., protein) on the nanometer scale.3 “You can almost look at anything, restricted to the surface,” said George Heath, a physicist at the University of Leeds who uses AFM to study cellular membranes.
The main AFM components that enable sample imaging at ultrahigh resolution include the following.
- Cantilever
- Tip
- Laser
- Detector
- Stage
- Piezoelectric scanner
Engineers utilize microfabrication techniques to develop sharp tips and extremely flexible cantilevers made of silica or silicon nitride, collectively known as probes, AFM sensors, or AFM tips.4 A probe functions as a force transducer.5 Its tip remains in contact or near contact with the sample surface as the attached cantilever moves across the sample.
During AFM imaging, scientists mount the sample on a piezoelectric scanner that enables 3D positioning with high resolution by generating an electrical charge in response to mechanical stress.6 A very small force generates between the tip and the sample surface when the sharp probe tip passes across the sample along the horizontal and vertical axes. AFM detects this force to determine changes in height or contours on the sample surface.
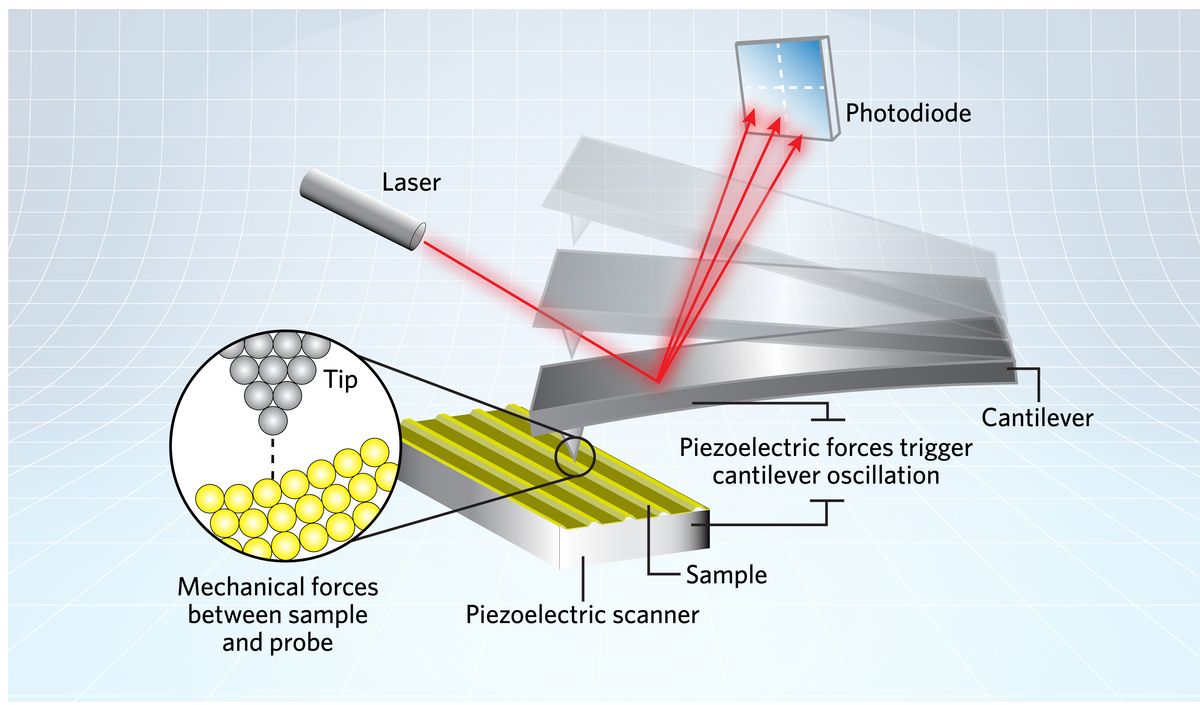
Force Measurements
When a probe comes closer to the sample surface, the cantilever bends upwards due to repulsive forces.1 Other surface forces generated are van der Waals, electrostatic, and steric/bridging forces. AFM detects the force generated during the cantilever deflection. A larger deflection indicates a higher force experienced by the probe.
The majority of AFM instruments use optical methods to assess cantilever deflection with high resolution. Optical methods use laser light projected on the free end of the cantilever, and a position sensitive detector (e.g., photodiode) captures the reflected beam.7
Probe Types
Different probe geometries are available, ranging from pyramidal to spherical. Scientists select probes based on the experiment type, probe resolution, durability, and conductivity requirements.8 For example, a sharp probe tip offers structural details, while a spherical probe provides mechanical measurements, such as how stiff or squishy a sample is.
Image resolution depends on the sharpness of the tip. “Imagine, if you are to feel across the surface with a tennis ball, if you have something like braille on the surface, you wouldn’t get any information,” explained Heath. “If you use a sharp pencil or a finger, then you will have the sharpness of the tip to get the resolution that you are interested in.” Concerning protein sample analysis, a sharp AFM tip provides information about the surface morphology, while a blunt probe images a protein without any structural details.
AFM Imaging Modes
The three common AFM imaging modes are contact, tapping, and non-contact.9 These modes vary based on the nature of tip motion.
Contact mode
Contact mode imaging is also known as static mode, which is the simplest AFM mode. In this mode, the probe is in physical contact with the sample surface during scanning. As the probe drags across the sample surface, the cantilever deflects based on the surface contours. Scientists use this mode to generate images at atomic resolution, particularly for samples that are not prone to damage by the sharp probe tip. Contact mode offers high speed scanning of rough surfaces that contain frequent changes in their vertical topography.
Tapping mode
Tapping mode is also known as intermittent or semi-contact mode. It provides high resolution imaging of samples that are otherwise difficult to image. For example, biological samples such as proteins are sometimes difficult to image because they might be loosely bound to the substrate or susceptible to damage.10
In this mode, the cantilever oscillates at its resonance frequency due to the presence of a piezoelectric crystal at its base that generate electric energy.11 When the probe scans the sample surface, the piezoelectric crystal triggers the cantilever to oscillate and the amplitude of oscillation ranges typically between 2nm and 100nm. Unlike contact mode, the probe tip in tapping mode is not dragged across the sample surface but is lifted off, therefore, reducing the risk of damaging the sample surface.
Non-contact mode
In non-contact mode, the probe is never in physical contact with the sample surface.12 Here, the tip hovers between 50Å and 150Å above the sample surface and vibrates at a particular frequency. When the probe comes in close proximity to the sample surface, its vibration frequency changes, which helps determine the sample topography. Scientists use this imaging mode when there is a risk of the probe tip altering the sample surface or tip sharpness, affecting image resolution.
AFM Helps Analyze Diverse Materials across Varied Research Fields
Researchers use AFM to study different materials in diverse science and technology fields. A key advantage of this analytical tool is its ability to perform imaging in varied mediums, including liquid, air, and ultrahigh vacuum, under room temperature, and in ambient pressure.13
AFM in semiconductors and polymer research
During semiconductor manufacturing, researchers experience multiple challenges to meet the increasing demand for microelectronic products that require shrinking device geometry.14 For microelectronic devices, the continual shrinking of metal-oxide-semiconductor field-effect transistor (MOSFET) geometry to nanometer scale could induce several leakage mechanisms including short-channel effects, gate dielectric direct tunneling, and band-to-band tunneling, causing circuit failure.
Automated AFM has played a significant role in meeting this challenge and characterizing narrower lines, holes, and trenches at 45nm nodes and beyond.15 AFM offers 3D images of a circuit’s surface and accurate critical dimension measurements. It enables analysis of faulty devices and conductive mapping of dielectrics.
Polymers are natural or synthetic substances containing large molecules linked together to form chains of repeating subunits.16 Over the years, scientists have used different polymers in many scientific areas and industries including as binders in batteries and composite materials for drug discovery. In polymer research, AFM detects the molecular stiffness of hyperbranched macromolecules, quantifies single polymer chain elongation, and assesses the friction of single polymers on surfaces.
AFM in biological and medical research
AFM provides information on biomechanical properties and nanostructural features of biological samples (e.g., cells and biomolecules).17 It helps determine the mechanical properties of the cell membrane, cell viscoelasticity, and cell stiffness. Scientists use AFM to analyze the nanostructure and nanomechanics of cells such as lymphocytes.18 In cancer research, high resolution images elucidate the ultrastructural and mechanical properties of tumor cells.19
In pharmaceutical research, AFM detects the interaction between supported lipid bilayers (SLBs) and drugs (e.g., azithromycin).20 It also characterizes microbial surfaces including their physical properties such as cell wall elasticity and adhesiveness that helps determine microbial resistance to drugs.
In vision research, scientists use AFM to study protein functions within cell membranes.21 The high resolution images help determine ligand-receptor interactions and illustrate nanoscale topography that influences vision. AFM images help correlate physical properties with disease states.
AFM Challenges and Future Opportunities
Many times, scientists obtain low resolution images while studying highly dynamic biomolecules such as proteins, which play an important role in medical research. Some biomolecules undergo rapid conformational changes multiple times, making them difficult to study via conventional AFM. A conventional AFM scans approximately two lines per second and it takes several minutes to image 500 lines and obtain a complete image. In contrast, high speed AFM (HS AFM) scans a thousand lines per second, which is ideal to analyze dynamic changes in biomolecules.22
For example, HS AFM is a fast and label-free technique that enables visualization of ion channels and transporters in a near native environment at an unprecedentedly high spatiotemporal resolution. “The advancement of high speed AFM allows you to scan very, very fast,” said Heath. This is important to study dynamic molecules that change shape and active state. HS AFM captures image series following the dynamics of biomolecules, which help researchers uncover the conformational changes in biological systems under varied physiological conditions. This understanding aids novel therapeutic designs. At present, scientists seek means to efficiently tackle the high volume of images generated by HS AFM to improve throughput.
Another challenge posed during AFM and HS AFM is sample preparation, particularly for new sample analysis. For example, it is essential to optimize surface concentration to avoid having a full layer of proteins in performing single particle analysis. Sample optimization is also essential for non-biological samples.
In the future, researchers aim to improve HS AFM speed, which could be beneficial for studying dynamic biomolecules with greater accuracy. AFM automation using artificial intelligence algorithms would further improve throughput and data processing of molecules with heterogeneous dynamics.
- Dufrêne YF. Atomic force microscopy, a powerful tool in microbiology. J Bacteriol. 2002;184(19):5205-5213.
- Giessibl FJ. Atomic force microscopy with qPlus sensors. MRS Bulletin. 2024;49:492–502.
- Joshua A, et al. Soft matter analysis via atomic force microscopy (AFM): A review. Appl Surf Sci Adv. 2023;17:100448.
- Wakayama T, et al. Micro-fabrication of silicon/ceramic hybrid cantilever for atomic force microscope and sensor applications. Sensors Actuat A-Phys. 2006;126(1):159-164.
- Fukuzawa K. AFM Probes. In: Bhushan, B. (eds)Encyclopedia of Nanotechnology. 2012;90-93.
- Gavara N. A beginner's guide to atomic force microscopy probing for cell mechanics. MRT. 2017;80(1):75-84.
- Herfst R, et al. Systematic characterization of optical beam deflection measurement system for micro and nanomechanical systems. Measurement. 2014;56:104-116.
- Xu K, Liu Y. Studies of probe tip materials by atomic force microscopy: a review. Beilstein J Nanotechnol. 2022;13:1256-1267.
- Dzedzickis A, et al. Characteristics and functionality of cantilevers and scanners in atomic force microscopy. Materials. 2023;16(19):6379.
- Whited AM, Park PSH. Atomic force microscopy: A multifaceted tool to study membrane proteins and their interactions with ligands. BBA-Biomembranes. 2014;1838(1):56-68.
- Alunda BO, Lee YJ. Review: Cantilever-based sensors for high speed atomic force microscopy. Sensors (Basel). 2020;20(17):4784.
- Baclayon M, et al. Sampling protein form and function with the atomic force microscope. Mol Cell Proteomics. 2010;9(8):1678-1688.
- Liu H, et al. A cantilever-based, ultrahigh-vacuum, low-temperature scanning probe instrument for multidimensional scanning force microscopy. Beilstein J Nanotechnol. 2022;13:1120-1140.
- Iwai H, et al. Challenges for future semiconductor manufacturing. IJHSES. 2006;16(01):43-8.
- Moon-Keun L, et al. Applications of AFM in semiconductor R&D and manufacturing at 45 nm technology node and beyond. Proc. SPIE 7272, Metrology, Inspection, and Process Control for Microlithography XXIII. 72722R. 2009.
- Maver U, et al. Polymer characterization with the atomic force microscope. In: InTech eBooks. 2013.
- Singh SB, et al. Protocol for measuring mechanical properties of live cells using atomic force microscopy. STAR Protoc. 2024;5(1):102870.
- Hu M, et al. Nanostructure and nanomechanics analysis of lymphocyte using AFM: From resting, activated to apoptosis. J Biomechs. 2008;42(10):1513-1519.
- Deng X, et al. Application of atomic force microscopy in cancer research. J Nanobiotechnology. 2018;16(1):102.
- Mingeot-Leclercq MP, et al. Atomic force microscopy of supported lipid bilayers. Nat Protoc. 2008;3:1654-1659.
- Last JA, et al. The applications of atomic force microscopy to vision science.Invest Ophthalmol Vis Sci. 2010;51(12):6083-6094.
- Heath GR, et al. Structural dynamics of channels and transporters by high-speed atomic force microscopy. Methods Enzymol. 2021;652:127-159.