ABOVE: © ISTOCK.COM, CHRISTOPH BURGSTEDT
There is only a certain amount of stress biological structures can withstand before they fall apart. Tissue fluids freeze into ice crystals when exposed to temperatures below −3 °C, for instance, and enzymes break down and become dysfunctional at extremely low temperatures. But there are organisms that are built to thrive in such harsh conditions. Some species of fungi, for instance, can survive the harsh weather in Antarctica, and scientists have spent years trying to figure out how. A study published in Science Advances on September 7 suggests that these polar organisms may have adapted due to tweaks to unstructured regions of their proteins.
The intrinsically disordered regions (IDRs) of proteins are the formless, liquidlike parts of proteins that lack the ability to fold into a functional shape and often react with RNA to form naked, or membraneless, cell organelles such as the nucleolus through a phenomenon known as liquid-liquid phase separation (LLPS).
See “These Organelles Have No Membranes”
The study shows that yeasts adapted to harsh cold experienced evolutionary changes in IDRs, which change how phase separation occurs. The researchers found that the structure of the IDRs in species adapted to polar regions is different from those in temperate areas.
The researchers stumbled on these differences while studying how transcription occurs in the cold. They were analyzing two primary components of the transcription system of RNA polymerase II multisubunit enzyme—carboxy-terminal domain (an IDR) and Ess1 prolyl isomerase—in five cold- and salt-adapted species of yeast isolated from the Arctic and Antarctica, when they noticed that the species’ carboxy-terminal domain’s (CTD’s) structure was slightly different than that of the model yeast species baker’s yeast (Saccharomyces cerevisiae).
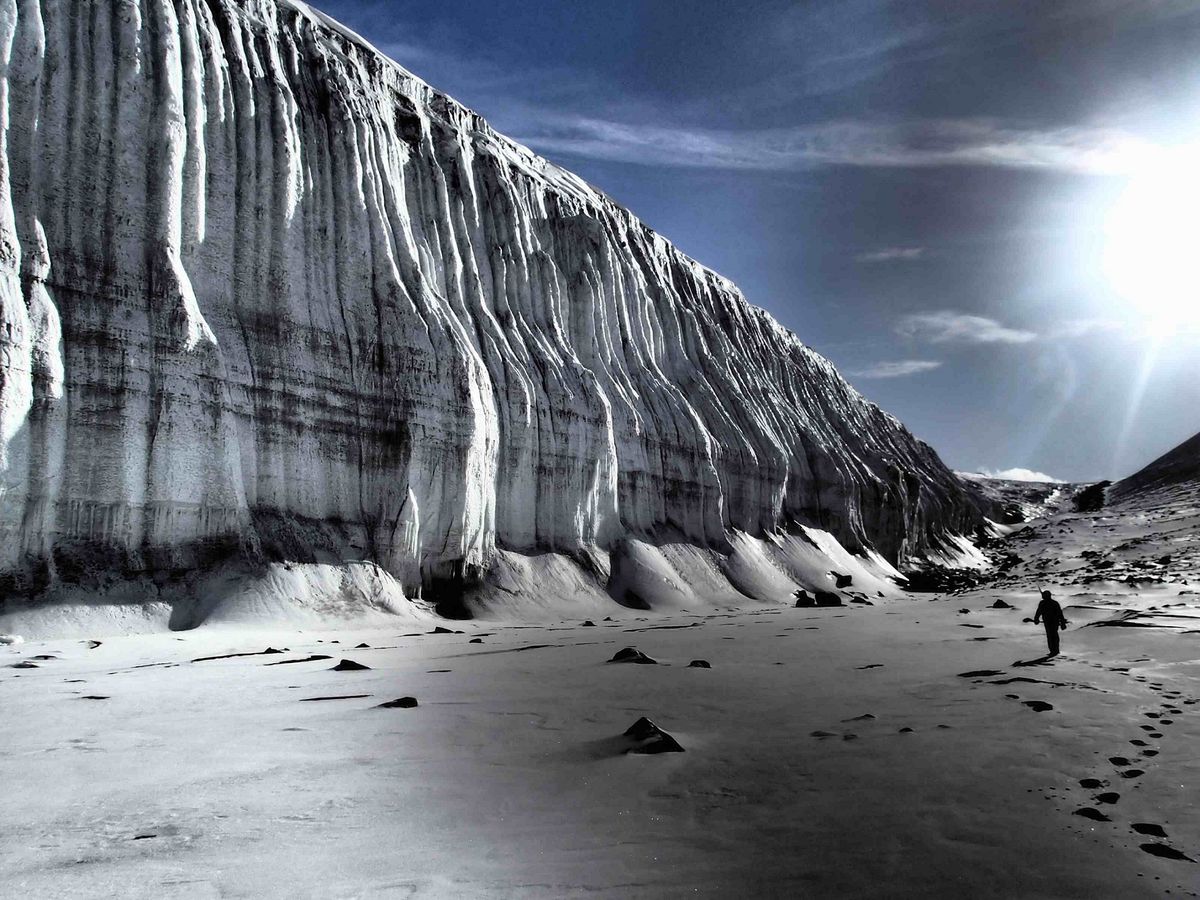
In baker’s yeast, the CTD consists of a repeating peptide sequence—YSPTSPS—whereas the polar yeasts’ repeating sequences diverge at positions one, four, and seven. Study coauthor Steven Hanes, a molecular geneticist at SUNY Upstate Medical University in New York, says that the repeating sequence is shared across vast clades of life and is nearly identical even in humans, so this divergence in cold-adapted yeasts was “extremely significant.” He and his colleagues were curious about why the polar yeasts’ CTDs manifest such differences and wondered if these CTDs would still function in baker’s yeast.
First, Hanes and colleagues deleted the gene that codes the CTD of baker’s yeast but kept the CTD intact by retaining the plasmid that expresses the enzyme Rpb1 (a subunit of RNA polymerase II), which kept the host cell alive. Next, they cloned a gene for polar yeasts’ CTD into a plasmid and transferred it into baker’s yeasts. They did this to test whether the divergent CTD would work when paired with the structured region of RNA polymerase II in baker’s yeast. The procedure was carried out at 18 °C and 30 °C to determine the effects of colder temperatures.
Hanes explains that if the cloned CTD gene are compatible with the host, they will push out the original plasmid from the host’s cell in favor of the new one. The degree to which the originals are lost will give an estimate of the cloned plasmid’s degree of compatibility with the model species.
The baker's yeast replaced its own plasmid with that of the various polar yeasts fairly well at 30 °C. But at 18 °C, the baker’s yeast containing CTDs from the Arctic fungi Wallemia ichthyophaga, Aureobasidium pullulans, and Hortaea werneckii lost only 0.2 percent, 13.6 percent, and 21.5 percent, respectively, while those that contained CTDs of the Antarctic fungi Dioszegia cryoxerica and Naganishia vishniacii did not lose any of the original plasmids. In contrast, the control lost 58 percent and 87 percent of the original plasmid at 18 °C and 30 °C, respectively. The researchers report that the CTD from polar yeasts did not work in baker’s yeast at 18 °C.
See “How a Bacterium Manages to Reproduce During Famine”
Hanes says that he suspected the differences may stem from the mechanisms of LLPS, as a previous study revealed CTDs can undergo the process. So the team checked if the CTDs from the cold-adapted yeast can also undergo this phenomenon.
The researchers bound polar CTDs with purified proteins in a test tube and checked for LLPS by observing the cloudiness of the solution—evidence that LLPS occurred—at different temperatures and salinity levels. Hanes and his team observed that the CTDs of these polar yeasts do undergo phase separation, but they do so differently than baker’s yeast. They noticed that the CTDs of species that were most compatible with S. cerevisiae at 18 °C exhibited high LLPS, while those that were not compatible showed none. The researchers attributed these differing properties to the divergence in the CTDs’ amino acid sequence, and they hypothesize that this divergence might promote cold and salt tolerance in the polar species.
Hanes says that the intrinsically disordered regions in the protein with more variable sequences are very adaptive to “selective pressures that would change the biophysical properties of how the proteins sort out within cells.” And this adaptation can change how, when, and where they undergo phase separation.
“We know that stress can induce phase separation by certain proteins, but what we are suggesting is that environmental tuning of phase separation allows organisms to tolerate temperature and other extreme conditions,” says Hanes.
Amy Gladfelter, a cell biologist at the University of North Carolina who studies phase separation and didn’t work on the new study, says that the results are “really suggesting that by looking at natural variation and . . . at how free-living yeast may adapt to extreme temperatures and also extreme salinity, [the research team] can find evidence of adaptation in sequences that are important to drive phase separation.”
Hanes tells The Scientist that the study has uncovered more questions than answers, but the team intends to resolve most of them, including the exact mechanism through which phase separation properties confer environmental tolerance, because this could help other microorganisms to survive harsh and changing climate conditions.